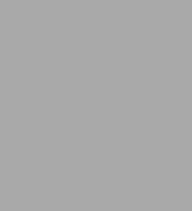
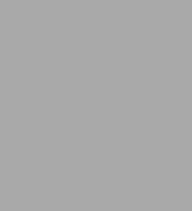
Paperback
-
PICK UP IN STORECheck Availability at Nearby Stores
Available within 2 business hours
Related collections and offers
Overview
A “mix of biology, history, medicine, and first-hand experience [that] is potent and irresistible,”* The Viral Storm: The Dawn of a New Pandemic Age shares information Wolfe uncovered on his groundbreaking and dangerous research missions in the jungles of Africa and the rain forests of Borneo to provide an in-depth exploration of how lethal viruses evolved alongside human beings; how illnesses like HIV, swine flu, and bird flu almost wiped us out in the past; and why modern life has made our species vulnerable to the threat of a global pandemic.
In a world where each new outbreak seems worse than the one before, Wolfe points the way forward, as new technologies are brought to bear in the most remote areas of the world to neutralize these viruses and even harness their power for the good of humanity. His provocative vision of the future will change the way we think about viruses, and perhaps remove a potential threat to humanity’s survival.
“An astonishingly lucid book on an important topic. Deeply researched, yet effortlessly recounted.”—*Siddhartha Mukherjee, Pulitzer Prize-winning author of The Emperor of All Maladies
Product Details
ISBN-13: | 9781250012210 |
---|---|
Publisher: | St. Martin's Publishing Group |
Publication date: | 10/16/2012 |
Pages: | 320 |
Sales rank: | 655,423 |
Product dimensions: | 5.58(w) x 8.06(h) x 0.82(d) |
About the Author
Read an Excerpt
Introduction
The village of Pang Thruk in the Kanchanaburi province of Thailand is like many in this part of the world—humid, lush, and spilling over with sounds of wildlife. Located in the west of the country near the border of Burma, Pang Thruk is home to about three thousand people whose livelihoods depend on the sugar and rice they grow. In December 2003, it was also home to Kaptan Boonmanuch, a six-year-old boy who would be among the first people to die of a brand-new human virus.
Kaptan loved riding his bicycle, climbing trees, and playing with his plastic toy Dalmatian that pulled three puppies in tiny brown wagons as it barked mechanically. Kaptan also enjoyed helping his family on the farm.
Nearly every family in Pang Thruk kept egg-laying chickens; some also kept roosters for cock fighting. Kaptan's aunt and uncle lived just down the road on their open-air farm of around three hundred chickens. Each winter in the village a few chickens would die from suspected infections or colds, but in December 2003 chicken deaths increased dramatically. That winter, as on many farms in this region, the chickens in Kaptan's uncle's farm suffered from severe diarrhea, strange behavior, and weakness. All of them either died naturally or were culled as a result of their illness—and Kaptan helped with the dead. A day or two before the New Year, according to reports, the boy carried one of the sick squawking chickens home. That walk home would have lasted no more than a few minutes.
A few days later Kaptan grew feverish. A clinic in the village diagnosed him with a cold, but after three days without improvement, his father, Chamnan, a rice farmer who worked part time as a driver, took him to a public hospital. X-rays revealed that the six-year-old had pneumonia, and he was kept in the hospital for observation. A few days more and Kaptan's fever spiked to a dangerous 105 degrees. His father paid the equivalent of thirty-six dollars for an ambulance to speed him to better care at Siriraj Hospital in Bangkok, more than an hour's drive away.
Upon arrival, Kaptan presented with shortness of breath and fever. Tests revealed that both lungs were affected with severe pneumonia, and the boy was transferred to the pediatric intensive care unit and put on a ventilator. A series of bacterial cultures tested negative, showing that the infection was likely caused by a virus. More detailed testing using a molecular technique called the polymerase chain reaction, or PCR, revealed that Kaptan was likely infected with an atypical type of influenza—perhaps one not yet seen (or seen widely) in humans.
After eleven days of illness, the boy's fever finally began to cool off. However, despite intensive care, his respiratory distress worsened. Just before midnight on January 25, physicians took Kaptan off the respirator. His lungs drowning in fluids, he became Thailand's first known death from H5N1, which would soon become known around the world as "bird flu."
As sad as Kaptan's death was—and the reports go on to describe the boy's funeral and the family's mourning in tragic detail—the reality is that children in the developing world die from diseases like this all the time. And infectious diseases, which scientists in the 1960s predicted would be eliminated in short order, remain some of the most important killers today. But when it comes to global risk, all deaths are not equal. Most deaths from infectious diseases are localized events that, while dire for the victims and their families, present limited risk to the planet as a whole. But some, like Kaptan's, signal a potentially world-altering event: the first human infection by an animal virus that may wipe out millions, or hundreds of millions, of people throughout the planet—permanently changing the face of humanity.
The main objective of my work is to hunt down these events—the first moments at the birth of a new pandemic—and then work to understand and stop them before they reach a global stage. Because pandemics almost always begin with the transmission of an animal microbe to a human, it's work that takes me all around the globe—from rain forest hunting camps of central Africa to wild animal markets of east Asia. But it also takes me to cutting-edge laboratories at the US Centers for Disease Control and Prevention (CDC) and disease outbreak control centers at the World Health Organization (WHO). Tracking down these potentially devastating bugs has led me to study how, where, and why pandemics are born. I work to create systems that can accurately detect pandemics early, determine their likely importance, and, with any luck, crush those that have the potential to devastate us.
As I've lectured on this work around the world and taught undergraduates in my virology seminar at Stanford University, it's hard to ignore a growing general interest in these topics. Everyone recognizes the raw power that pandemics have to sweep through human populations and seemingly kill indiscriminately. Yet, given the importance of these events, large questions remain remarkably opaque:
How do pandemics start?
Why are we now plagued with so many pandemics?
What can we do to prevent pandemics in the future?
This book is my attempt to answer these questions—an effort to assemble the pieces of the pandemic puzzle.
Part I, "Gathering Clouds," introduces our main character, the microbe, [1] and delves into the history of our relationships with these organisms. It explores the vast world of microbes, putting those that threaten us in their proper perspective. It details some of the most significant events in the evolution of humans and our ancestors and works to develop the often-spotty historical data into a set of hypotheses on how the events influenced our interactions with microbes.
Part II, "The Tempest," examines how contemporary human populations have grown so exquisitely susceptible to pandemics and what the future years will hold for pandemic diseases. Part III, "The Forecast," describes the fascinating new world of pandemic prevention and introduces a new crop of scientists eager to help create a virtual global immune system that will stop pandemics before they become planetary nightmares. Along the way, we will journey to remote hunting villages in central Africa, investigate malaria in wild orangutans in Borneo, learn how cutting-edge genetic sequencing tools will change the way that we discover completely novel viruses, and see how Silicon Valley companies may forever transform the way that we conduct surveillance aimed at finding the next major outbreak.
At this point you may be asking yourself how someone ends up devoting his career to the study of plagues. Is it a desire to save the world? Or perhaps it's the scientific thrill of discovering completely unknown, invisible beings with the potential to wipe out large swaths of humanity. Maybe it's the desire to understand in detail one part of humans' intricate ecology. Or it's an urge to explore the exotic locations on Earth where these novel viruses often appear. But while my life is now consumed with trying to understand and stop pandemics, that's not how it's always been. My work with microbes actually started as a minor footnote to a study I wanted to conduct among wild chimpanzees in central Africa.
As a young child, I acquired what would become a lifelong interest in apes when I watched a National Geographic documentary explaining how humans are more closely related to apes than we are to monkeys. A family tree with humans and apes as siblings (and monkeys as distant cousins) was entirely inconsistent with my memories of seeing these creatures locked up together in the "monkey house" at the Detroit Zoo. We humans were outside the cages, and the rest of them were inside. The idea that apes and humans were closely related certainly struck a chord with me. According to my father, I spent some days after the documentary playing the part: walking around the house on all fours, trying to communicate without language, and otherwise working to bring out my inner ape.
My fascination with apes evolved from a childish curiosity to an intellectual interest in what our closest relatives had to tell us about ourselves. What began as a broad interest in apes as animals became a more specific interest in chimpanzees and their less acknowledged brethren, the bonobos—the two ape species that share our own particular branch of the tree of life. How did the years of separation since our last common ancestor with these two kindred ape species shape our minds, our bodies, and our worlds? What features remained the same in all of us?
Along with the intellectual interest, I had a growing desire to see these apes living in their natural environment. This desire required tracking them down in the rain forests of central Africa to see for myself what they were really like. So when choosing among doctoral programs, I settled on one at Harvard where I would work with Richard Wrangham and Marc Hauser, two prominent primatologists. I would spend many months during my first year of doctoral work arguing why they should let me study the troops of wild chimpanzees that Wrangham had worked with for years in the Kibale Forest in southwest Uganda.
I proposed a study to document self-medicating behavior of the Kibale chimpanzees. The idea that these animals consumed plants with specific medicinal chemicals as a way of fighting against their own infectious diseases was still just an hypothesis at the time, and an intriguing one. I'd explored this idea the previous year while studying at Oxford and working on an exhibit about animal self-medication at the Oxford University Museum of Natural History.
The Oxford University Museum is a magnificent nineteenth-century building constructed in the architectural style of a Gothic cathedral but with massive iron supports mimicking the skeleton of a mammal, emphasizing that it was a church of natural history rather than religion. It houses unique collections, including some of the beetles collected by Charles Darwin on his celebrated voyage on the Beagle. It had been home to the famous Huxley-Wilberforce debate on natural selection in 1860 seven months after the publication of Darwin's pivotal book On the Origin of Species. It's a perfect location to ponder the place of humans in the natural world. The work I did under the supervision of the eminent evolutionary biologist W. D. Hamillton and his colleague Dale Clayton, an expert in behaviors animals used to rid themselves of parasites, revealed that self-medication was widespread in the animal kingdom. Animals as disparate as wasps and Kodiak bears utilized the chemical defenses of plants to help rid themselves of their natural pests.
As I began my work in Uganda to study chimpanzees, my professors cautioned me that any convincing proof that chimpanzees were medicating themselves with plants would require an understanding of the infectious diseases they were treating. Unless I could show that the use of the purported medicines decreased the burden of disease, my results would be speculative at best. I needed to understand what infectious diseases plagued the chimpanzees. I knew little about microbes, so I approached Andy Spielman, a professor at Harvard's School of Public Health and one of the few people at the time focused on understanding the ecology of microbes in nature. Despite his lab full of fellows and students and his focus on North America rather than the wilds of Africa or Asia, he kindly took me under his wing. Thus began my research on what was known about the infections of chimpanzees. Once I began thinking about microbes I never looked back. And central to my studies would be the viruses.
Viruses evolve more rapidly than any organism on the planet, yet we understand less about them than any other form of life. [2] The study of viruses provides a scientist with the opportunity to discover new species and catalog them in a way reminiscent of the world of the nineteenth-century naturalist, which had so fascinated me during my time at Oxford. A scientist can productively spend an entire career looking for new species of primate and never find one, but new viruses are discovered every year. They also have exceedingly short generations, so we can watch them evolve in real time—an ideal system for someone interested in understanding the process of evolution. Perhaps best of all from the perspective of a young scientist, there was important and urgent low-hanging fruit in this discipline: some of these viruses kill us. Thus new discoveries need not only lead to an improved understanding of nature, but they can also have important and rapid applications for controlling human disease.
Controlling the spread of human disease was at the forefront of public health efforts in early 2004 when the news broke of Kaptan's death from H5N1. His death was the first confirmed mortality from this virus, the so-called bird flu, in Thailand. The truth is that while they may jump to us via other animals, all human influenza viruses ultimately originate in birds, so the popular designation of the virus as "bird flu" can irritate scientists. Yet within a month that name would become a mainstay of news shows and a topic of discussion for people throughout the world.
The scientific name for the virus that killed Kaptan, HPAIA (H5N1), is quite descriptive for virologists. It signifies that the virus is a highly pathogenic avian influenza A-type virus and provides the particular hemagglutinin (H) and neuraminidase (N) protein variants particular to this virus strain. But its true significance is actually much more straightforward.
H5N1 is important because it kills remarkably effectively. The virus's case fatality rate, or the percentage of infected individuals that die, is around 60 percent. For a microbe, that's incredibly deadly. As a comparison, we can look back to the devastating 1918 influenza pandemic. While estimates for the 1918 pandemic are imperfect, it is thought around fifty million people died. That's the equivalent of 3 percent of the entire human population at that time, an almost unimaginable catastrophe. To put this in context, more people died from the 1918 influenza pandemic than the total number of soldiers thought to have died in battle during all twentieth-century wars combined. More deaths caused by a simple virus, less than one hundred nanometers in diameter and with only a paltry eleven genes, than were caused by all the battles in WWI, WWII, and all of the other wars in our last war-riddled century. Despite the enormity of the 1918 plague, the highest estimates for its case fatality rate are in the range of 20 percent, and it was almost certainly much lower than that; more careful estimates suggest around 2.5 percent. [3] Recall that H5N1 had a 60 percent fatality rate, far greater than that of the influenza virus that caused the 1918 pandemic.
But while deadliness is an important, dramatic, and ongoing obsession of the media, it is only one piece of the puzzle for microbiologists. In fact, some microbes kill virtually all people they infect: a perfect 100 percent mortality rate. And yet such microbes do not necessarily represent critical threats to humanity. Viruses like rabies, which naturally infect a number of mammal species, and the herpes B virus, which naturally infects some species of Asian monkey, will kill all the people they infect. [4] Yet unless you're someone who is exposed to rabid animals or works with Asian monkeys, these microbes do not represent a major concern for you. That's because they don't have the capacity to spread from person to person. In order to be catastrophic, a microbe needs both the potential to harm or kill and the potential to spread.
In early 2004 there was no way to know how efficiently H5N1 would spread. Since it comes from a class of viruses that often do spread, the influenza viruses, the possibility was there. And if H5N1 were to spread in the same way that the 1918 influenza virus spread, it would create a calamity unlike any seen before in human history.
As impressive as H5N1 is at killing, H1N1, the so-called swine flu, [5] is equally impressive in its capacity to spread. While no one knows exactly when the H1N1 pandemic began, by August 2009, less than a year after it was first recognized, the WHO announced estimates suggesting that the virus could eventually infect over two billion people, roughly a third of the entire human population. The natural drama of this would be hard to overstate. While less visually dramatic than other forms of natural disaster, the ability of this phenomenon to touch people everywhere on the planet made it a powerful force of nature. A virus that likely infected few people in early 2009 moved around the globe to inhabit a sizable percentage of the entire population of the human species in less than a year. This occurred despite the best efforts of global public health infrastructures—structures that we tend to be very proud of and feel protected by. Yet while the case fatality rate of H1N1 is estimated to be well below 1 percent, paling in comparison to H5N1, the sheer number of people it infected has made it a real global killer. One percent of two billion is a lot of lives.
To help us understand the real threat of an outbreak, we turn to a concept in epidemiology called R0, or the basic reproductive number. For any epidemic, R0 is the the average number of subsequent infections that each new case results in (in the context of a population with no prior immunity and no control efforts). If, on average, each case of a new epidemic leads to more than one subsequent infection, the new epidemic has the potential to grow. If, on average, each case leads to less than one subsequent infection, it will peter out. The elegant concept of R0 helps epidemiologists distinguish between epidemics likely to "go viral" and those likely to go extinct. It's basically a measure of scalability.
Risk interpretation is not a trivial matter, for either the public or the policy makers. In the case of H1N1 or H5N1, the potential costs of not rushing to develop a vaccine or working to decrease transmission could have been global and catastrophic.
Critically, microbes are dynamic—they do not exist in stasis. If H5N1, the deadly bird flu, gains the right combination of genetic mutations it needs to spread effectively, the results will be destructive in a way that, however less visually dramatic, will make even the most deadly earthquakes seem like a walk in the park. And if H1N1, the rapidly spreading swine flu, were to increase in virulence even minimally, its potential to kill would be striking. Neither scenario is implausible. As chapter 1 will explore in detail, influenza and a range of other viruses have an incredible capacity to negotiate the environment of their human hosts. They mutate rapidly, even swapping genes among themselves, a process referred to as reassortment.
It is just such reassortment that concerned me, and other scientists, in 2009. As the H1N1 virus spread explosively around the world, there was a nontrivial chance that it would run into H5N1 in people or animals, setting the stage for a potentially cataclysmic series of events. These are the kind of events we work to uncover early before they spread. When infected simultaneously with both of these influenza viruses, a particular human or animal could become a potent mixing vessel, providing the perfect opportunity for the bugs to swap genes. How would this happen? In a sort of sexual reproduction, H5N1 and H1N1 could produce mosaic daughter viruses with some of the genes of one virus and some of the genes of the other. Such reassortment events can occur in individuals infected with multiple similar viruses. In the case of H1N1 and H5N1, if that mosaic daughter virus inherited the potential to spread from its H1N1 parent and the deadliness of its H5N1 parent, the resulting virus would be both highly transmissible and highly fatal—exactly the formula for global impact that we most fear.
A mad rush to respond to pandemics has been the mainstay of global public health for the last one hundred years. Now a small but vocal group of scientists and I have begun to argue that we must do better than just respond to pandemics by scrambling for vaccines, developing drugs, and modifying behaviors. This traditional approach has proved a failure for human immunodeficiency virus (HIV), which nearly thirty years after its discovery continues to spread, currently infecting over thirty-three million people at last count.
But what if we had been able to catch HIV before it spread? This virus was in humans for over fifty years before it spread widely. It spread for another twenty-five years before French scientists Françoise Barré-Sinoussi and Luc Montagnier, who would go on to win a much-deserved Nobel Prize for their work, finally discovered it. How different would the world be had we stopped it before it left central Africa?
The idea that we might one day predict pandemics is a new one. The first time I heard someone discuss it was around ten years ago in the Johns Hopkins office of Don Burke, a retired medical colonel and world-renowned virologist from the Walter Reed Army Institute of Research (WRAIR) who had devoted his life to more traditional approaches to control disease before he took on a professorship at Johns Hopkins University's Bloomberg School of Public Health. Don had hired me as a postdoctoral fellow at Hopkins a few years earlier when I was finishing up my doctoral work in the rain forests of north Borneo studying the ways that mosquitoes and other blood-feeding insects helped microbes move between primate species.
Unable to track me down himself, Don had managed to find my mother in Michigan and gave her a call. My mother, whom I would speak to infrequently on trips from our forest research station, scolded me, saying that a "general" from the US military had called her. She asked what kind of trouble I had managed to get myself into. Fortunately, all Don wanted was to see if I'd help him set up a project in central Africa to understand how viruses emerge from animals into human populations.
During the years that followed, in addition to the long, slogging work of building research capacity to catch new microbes in central Africa and Asia, Don and I engaged in hours of conversation in the field and in his office in Baltimore. We made numerous beer bets on scientific problems and asked hard questions about the future of our field. I remember the day when I first heard Don suggest that the future would include not only response to pandemics but prediction. It seemed a bold but logical idea, and we quickly moved toward thinking about ways that it could actually happen. Those early conversations formed the foundation of the work my colleagues and I conduct now, setting up and running listening posts at microbial hot spots around the world aimed at catching new microbes locally before they become global pandemics.
Among the things we listen for are novel influenza viruses, like H5N1 and H1N1. Unfortunately, the world too easily becomes complacent to threats like H5N1 and H1N1. These and other threats fade quickly from the media's attention. Most of the world doesn't think about either of these viruses seriously. Yet neither virus has gone extinct, and the threat is perhaps as great now as it was when they were each first noticed. Both viruses continue to infect human populations. For example, in 2009, years after the media forgot about the virus, H5N1 caused at least seventy-three laboratory-confirmed cases. This is almost certainly a substantial underestimate of the number of actual cases and does not differ notably from the number of confirmed cases from previous years. H1N1 cases continue to spread as well. We have detected them even in the most remote forest areas where we "listen."
Amazingly, in a time when we can sequence an entire human genome for under ten thousand dollars and build massive telecommunications infrastructures that will soon make cell phones available to most people on the planet, we still understand surprisingly little about pandemics and the microbes that cause them. We know even less about how to predict or prevent pandemics before they spread from small towns to cities and the rest of the world. As I'll argue in part II, pandemics will increase in frequency in the coming years as the connections between human populations and the animals in our world continue to grow. Whether it's a mosaic virus with the deadliness of H5N1 and the potential to spread like H1N1, a resurgent SARS, a new retrovirus like HIV, or perhaps most frighteningly, a completely novel microbe that blindsides us, microbial threats will grow in the coming years in their ability to plague us, kill people, destroy regional economies, and threaten humanity in ways more severe than the worst imaginable volcanoes, hurricanes, or earthquakes.
A storm is brewing. The objective of this book is to understand this coming storm—to explore the nature of pandemics, to understand where they come from and where they are going. But it will not paint a completely grim picture. In the one hundred years since we first discovered viruses, humans have come a long way in understanding them. Much hard work remains. Yet if we do it well, we will harness the numerous technological advances of our time that provide tools to predict pandemics—just as meteorologists predict the course of hurricanes—and ideally prevent them from occurring in the first place. This is the Holy Grail of modern public health, and in the coming pages, I will argue that it is within our grasp.
PART I
Gathering Clouds
1
The Viral Planet
Martinus Beijerinck was a serious man. In one of the few images that remain of him, he sits in his Delft laboratory in the Netherlands, circa 1921, just a few days before his reluctant retirement. Bespectacled and in a suit, he's presumably as he'd like to be remembered—among his microscopes, filters, and bottles of laboratory reagents. Beijerinck had some peculiar beliefs, including the idea that marriage and science were incompatible. According to at least one account, he was verbally abusive to his students. While rarely remembered in the history of biology, this strange and serious man conducted the pivotal studies that first uncovered the most diverse forms of life on Earth.
Among the things that fascinated Beijerinck in the late nineteenth century was a disease that stunted the growth of tobacco plants. Beijerinck was the youngest child of Derk Beijerinck, a tobacco dealer who went bankrupt due to crop losses caused by this blight. Tobacco mosaic disease causes discoloration in young tobacco plants, leading to a unique mosaic pattern on leaves and radically slowing the growth of the adult plants. As a microbiologist, Beijerinck must have been frustrated by the unclear etiology of the disease that had wiped out his father's business. Despite the fact that it spread like other infections, microscopic analysis did not reveal a bacterial cause of disease. Curious, Beijerinck subjected the fluids of one of the diseased plants to intense filtration using a fine-grained porcelain filter. He then demonstrated that even after such filtration the fluids retained their capacity to infect healthy plants. The tiny size of the filter meant that bacteria, the usual suspects for transmissible disease at the time, would be too large to pass through. Something else must have caused the infection—something unknown and considerably smaller than everything else recognized to be alive in his time.
Unlike his colleagues, many of whom believed a bacteria would emerge as the cause, Beijerinck concluded that a new form of life must cause tobacco mosaic disease. [6] He named this new organism the virus, a Latin word referring to poison. The word virus had been around since the fourteenth century, but his use was the first to link it to the microbes to which it refers today. [7] Interestingly, Beijerinck referred to viruses as "contagium vivium fluidum," or "soluble living agent," and felt they were likely fluid in nature. That is why he used the term virus—or poison—to denote its "fluidity." It wasn't until later work with the polio and foot-and-mouth-disease viruses that the particulate nature of viruses was confirmed.
In Beijerinck's time a new microscopic perspective began revealing itself to scientists. Looking through microscopes and applying gradually smaller filters, these microbiologists realized something that continues to amaze us today: shielded from our human-scaled senses is a wide, teeming, startlingly diverse, unseen world of microbial life.
I teach a seminar at Stanford called Viral Lifestyles. The title was meant to evoke curiosity among prospective students but also describe one of the course's objectives: to learn to envision the world from the perspective of a virus. In order to understand viruses and other microbes, including how they cause pandemics, we need to first understand them on their own terms.
The thought experiment that I give my students on the first day is this: imagine that you have powerful glasses allowing you to perceive any and all microbes. If you were to put on such magical bug-vision specs, you would instantly see a whole new, and very active, world. The floor would seethe, the walls would throb, and everything would swarm with formerly invisible life. Tiny bugs would blanket every surface—your coffee cup, the pages of the book on your lap, your actual lap. The larger bacteria would themselves teem with still smaller bugs.
This alien army is everywhere, and some of its most powerful soldiers are its smallest. These smallest of bugs have integrated themselves, quite literally, into every stitch of the fabric of earthly life. They are everywhere, unavoidable, infecting every species of bacterium, every plant, fungus, and animal that makes up our world. They are the same form of life that Beijerinck found in the late days of the nineteenth century, and they are among the most important of the microbial world. They are viruses.
Viruses consist of two basic components, their genetic material—either RNA or DNA—and a protein coat that protects their genes. Because viruses don't have the mechanisms to grow or reproduce on their own, they are dependent on the cells they infect. In fact, viruses must infect cell-based life forms in order to survive. Viruses infect their host cells, whether they are bacterial or human, through the use of a biological lock-and-key system. The protein coat of each virus includes molecular "keys" that match a molecular "lock" (actually called a receptor) on the wall of a targeted host cell. Once the virus's key finds a matching cellular lock, the door to that cell's machinery is opened. The virus then hijacks the machinery of that host cell to grow and propagate itself.
Viruses are also the smallest known microbes. If a human were blown up to the size of a stadium, a typical bacterium would be the size of a soccer ball on the field. A typical virus would be the size of one of the soccer ball's hexagonal patches. Though humans have always felt virus's effects, it's no wonder it took us so long to find them.
Viruses, the most diverse forms of life, remained completely opaque to humans until a meager one hundred years ago with Beijerinck's discovery. Our very first glimpses of bacteria came a little under four hundred years ago when Antonie van Leeuwenhoek adapted the looking glasses of textile merchants to create the first microscope. With it, he saw bacteria for the first time. This finding represented such an incredible paradigm shift that it took the British Royal Society another four years before it would accept that the unseen life forms were not merely artifacts of his unique apparatus.
Our scientific understanding of unseen life has proceeded pitifully slowly. Compared to some of the other major scientific breakthroughs over the last few thousand years, our understanding of the dominance of unseen life occurred only recently. By the time of Jesus, for example, we already understood critical elements of how the Earth rotated, its rough size, and its approximate distance to the sun and moon—all fairly advanced elements in understanding our place in the universe. By 1610 Galileo had already made his first observations using a telescope. Van Leeuwenhoek's microscope came fifty years after that.
It is hard to overstate the paradigm shift that van Leeuwenhoek's discovery represents. For thousands of years humans had recognized the existence of planets and stars. Yet our understanding of unseen life and its ubiquity began only a few hundred years ago with the invention of the microscope. The discovery of novel life forms continues to this day. The most recent novel life form to be uncovered is the unusual prion, whose discovery was acknowledged with a Nobel Prize in 1997. Prions are an odd microscopic breed that lack not only cells but also DNA or RNA, the genetic material that all other known forms of life on Earth use as their blueprint. Yet prions persist and can be spread, causing, among other things, mad cow disease. We would be arrogant to assume that there are no other life forms remaining to be discovered here on Earth, and they are most likely to be members of the unseen world. [8]
We can roughly divide known life on Earth into two groups: noncellular life and cellular life. The major known players in the noncellular game are viruses. The dominant cellular life forms on Earth are the prokaryotes, which include bacteria and their cousins, the archaea. These life forms have lived for at least 3.5 billion years. They have striking diversity and together make up a much larger percentage of the planet's biomass than the other more recognizable cellular forms of life, the eukaryotes, which include the familiar fungi, plants, and animals.
Another way of categorizing life is this: seen and unseen. Because our senses detect only the relatively large things on Earth, we are parochial in the way that we think about the richness of life. In fact, unseen life—which combines the worlds of bacteria, archaea, and viruses as well as a number of microscopic eukaryotes—is the truly dominant life on our planet. If some highly advanced extraterrestrial species were to land on Earth and put together an encyclopedia of life based on which things made up most of Earth's diversity and biomass, the majority of it would be devoted to the unseen world. Only a few slender volumes would be dedicated to the things we normally equate with life: fungi, plants, and animals. For better or worse, humans would make up no more than a footnote in the animal volume—an interesting footnote but a footnote at best.
Global exploration to chart the diversity of microbes on the planet remains in its infancy. Considering viruses alone gives some sense of the scale of what's unknown. It's thought that every form of cellular life hosts at least one type of virus. Essentially—if it has cells, it can have viruses. Every alga, bacterium, plant, insect, mammal. Everything. Viruses inhabit an entire microscopic universe.
Even if every species of cellular life harbored only one unique virus, that would by definition make viruses the most diverse known life forms on the planet. And many cellular life forms, including humans, harbor a range of distinct viruses. They are found everywhere—in our oceans, on land, deep underground.
The dominant forms of life on our planet, when measured in terms of diversity, are unambiguously microscopic.
The largest virus to be discovered is the still microscopic six-hundred-nanometer Mimivirus—viruses are by nature tiny. But the sheer number of viruses in our world leaves a significant biological impression. A groundbreaking paper published in 1989 by Oivind Bergh and his colleagues at the University of Bergen in Norway found up to 250 million virus particles per milliliter of seawater, using electron microscopy to count the viruses. Alternate, more comprehensive measurements of the biomass of viruses on Earth are even more unimaginably outsized. One estimate suggests that if all the viruses on Earth were lined up head to tail the resulting chain would extend 200 million light years, far beyond the edge of the Milky Way. Though often thought of as a pesky irritant or blight, viruses actually serve a role that goes far beyond, and has a much greater impact than, what was previously understood—a role that scientists are only just beginning to comprehend.
It's true that in order to complete their life cycle, viruses have to infect cellular forms of life, but their role is not necessarily destructive or harmful. Like any major component of the global ecosystem, viruses play a vital role in maintaining global equilibrium. The 20 to 40 percent of bacteria in marine ecosystems that viruses kill every day, for example, serves a vital function in the resulting release of organic matter, in the form of amino acids, carbon, and nitrogen. And though studies in this area are few, it is largely believed that viruses, in any given ecosystem, play the role of "trust busters"—helping to ensure that no one bacterial species becomes too dominant—thereby facilitating diversity.
Given the ubiquity of viruses, it would be surprising indeed if they were relegated to a destructive role. Further studies will likely reveal the profound ecological importance of these organisms not just in destroying but also in benefiting many of the life forms they infect. Since Beijerinck's discovery, the vast majority of research conducted on viruses has understandably focused on the deadly ones. In the same way, we know much more about venomous snakes, despite the fact that they represent a startlingly small percentage of snake diversity. As we consider the frontiers of virology in part III, we will explore the potential benefits of viruses in detail.
Viruses infect all known groups of cellular life. Whether a bacterium living in the high-pressure depths of the planet's upper crust or a cell in a human liver, for a virus, each is just a place to live and produce offspring. From the perspective of viruses and other microbes, our bodies are habitats. Just as a forest provides a habitat for birds and squirrels, our bodies provide the local environment in which these beings live. And survival in these environments presents a range of challenges. Like all forms of life, viruses compete with each other for access to resources.
Viruses face constant pressure from our immune systems, which have multiple tactics to block their entrance into the body or disarm and kill them when they manage to get in. They face constant life choices: should they spread, which risks capture by our immune systems, or remain in latency, a form of viral hibernation, which can provide protection but sacrifices offspring.
The common cold sore, caused by the herpes simplex virus, illustrates some of the challenges that viruses face in negotiating the complex habitats of our bodies. These viruses find refuge in nerve cells, which because of their privileged and protected positions in our bodies do not receive the same level of immune attention as the cells in our skin, mouth, or digestive tract. Yet a herpes virus that maintained itself within a nerve cell without spreading would hit a dead end. So herpes viruses sometimes spread down through the nerve cell ganglions to the face to create virus-loaded cold sores that provide them a route to spread from one person to the next.
How viruses choose when to launch themselves remains largely unknown, but they almost certainly monitor the environmental variables of their world when making these decisions. Many of the adult humans who are infected with herpes simplex virus know that stress can bring on cold sores. Some also have noted anecdotally that pregnancy seems to bring on active infections. While still speculation, it would not be surprising if viruses responded to environmental cues indicating severe stress or pregnancy by activating. Since severe stress can indicate the possibility of death, it may be their last opportunity to spread—a dead host is also a dead virus. A pregnancy, on the other hand, presents the opportunity for spread either through genital contact with the baby during childbirth or during the kissing that inevitably follows the birth of a baby.
Transmission from host to host is such a fundamental need for infectious agents that some take it a step further. The incredible malaria parasite Plasmodium vivax hibernans goes so far as to keep a calendar of sorts. Many times larger than herpes simplex virus, parasites like malaria are infectious agents like viruses and bacteria but are in the eukaryotes class, and so are more closely related to animals than they are to the others. Spread by mosquitoes, P. vivax hibernans persists in arctic climates. In these cold locations, it can only infect mosquitoes seasonally during the brief period each summer when the insects hatch. Rather than wasting energy producing offspring all year, the malaria parasite lies dormant for most of the year in the human liver but, during summer, bursts to life, generating its spawn of malaria offspring that spread through an infected person's blood. While it's still unclear exactly what triggers the relapse, recent studies suggest that it might be the bites of mosquitoes themselves that provide an indication that the season for spreading has begun.
The careful timing that viruses and other microbes use when choosing to spread does not differ from the choices that other organisms make. Whether the timing of fruiting in a tropical fruit tree or the timing of mating in water buffalo, living things that time their reproduction appropriately have more successful offspring. This means the traits for accurately timing reproduction will persist and diversify. And how microbes time their growth within our bodies also has a major impact on illness.
The majority of microbes that cause infection in humans are relatively harmless, but some have a striking capacity to make us sick. This can sometimes be expressed in the form of, say, a common cold (caused by a rhinovirus or adenovirus) but can also manifest itself in life-threating illnesses such as smallpox.
Deadly microbes are a consistent challenge to evolutionary biologists because of their paradoxical habit of eviscerating habitats upon which they depend for their own survival. It's analogous to a bird destroying the forest in which it and its descendants live. Yet the process of evolution occurs largely at the level of the individual or even the gene. Evolution does not proceed with forethought, and there's nothing to stop a virus from spreading in such a way that leads to a dead end. Such virally induced extinction events have undoubtedly occurred throughout the history of interactions with microbes, no matter the ultimate cost for virus or host.
More central from the perspective of a virus is the impact of disease on transmission. As we learned in the introduction, on average, each germ must infect at least one new victim for every old one who either dies or recovers and purges himself of the microbe in order to avoid extinction. This is the rule of the basic reproductive number, or R0. If the average number of new victims per old victims drops to less than one, then the spread of the microbe is doomed. Since microbes generally can't walk or fly from one host to the next they often strategically alter their host to help in their spread. From the perspective of a bug, a symptom can be an all-important means of enlisting our help in moving itself around. Microbes often make us cough or sneeze, which can permit them to spread through our exhaled breath, suffer from diarrhea, which can spread microbes through local water supplies, or cause open sores to appear on our skin, which can spread through skin-to-skin contact. In these cases it's obvious why a microbe would trigger these generally unpleasant symptoms. Unpleasant symptoms are one thing, but killer microbes are quite another.
Keeping its host alive and pumping out new microbes would seem to be an ideal plan for a bug. And some bugs do certainly employ such a strategy. Human papillomavirus, or HPV, infects around 50 percent of sexually active adults at some point during their lifetimes. It currently infects around 10 percent of people on the planet, a staggering 650 million people. And while a few strains of HPV cause cervical cancer, most do not. Those strains that do kill their hosts infect them for many years before showing any symptoms at all. Even if the current vaccines that protect against the cancer-causing HPV variants were deployed universally, harmless HPV strains would continue to circulate at huge levels with an impact no greater than occasional if unsightly warts. These viruses can spread effectively without killing. Yet other bugs kill with startling efficiency.
Bacillus anthracis, a bacterial pathogen of grazing animals like sheep and cattle that occasionally infects humans, causes anthrax infection, which kills quickly and effectively. Following ingestion of anthrax spores during grazing, anthrax reactivates and spreads rapidly throughout the animal, often killing it in short order. But this dead host is by no means a dead end. After using the energetic resources of its dying host to replicate in massive numbers, anthrax simply goes back into spore form. Wind, a common feature of the grassy plains of the grazing hosts, then spreads the spores throughout the environment, where they can wait for new prospective victims to arrive. In the case of anthrax, creating hardy spores frees the bug from any negative consequences of its destruction.
Such situations are not limited to spore-forming bacteria. The cholera bacterium, which gives us diarrhea, and the smallpox virus, which causes severe viral disease, both kill in only days or weeks. But before the deaths take place, the deadly symptoms spread trillions of microbes to potential new victims. Human deaths, while unfortunate to us, represent a mere consequence of the conditions the bugs need to get to their next hosts.
From the perspective of a bug the impact on its host is only measured in its ability to survive and reproduce. And altering our physical bodies is just the beginning. Some microbes also influence our behavior, effectively making us zombies acting in their benefit. One of the most striking examples comes from a feline parasite, Toxoplasma gondii. While toxo, as parasitologists refer to it, can infect a range of mammals from humans to rodents, its life cycle cannot be completed until it lands in a cat. This parasite has found a frighteningly effective way to get home when it ends up in the wrong mammal. Careful studies have documented how the parasite spreads to the nervous system of infected, unsuspecting rodents and hijacks their brains. Sometime after infection, having spent much of their life steering clear of cats, mice begin to see them as positively enticing. This fatal attraction leads to a dead mouse, but also a toxo cyst that has the potential to complete its life cycle in the newly infected, not to mention, satiated, cat.
Truly deadly diseases must strike a balance between the likelihood of causing death in its victim once the victim is infected and their efficacy in terms of allowing the victim to spread the disease to others. You can't generally have your cake and eat it, too—producing many microbes in a host increases the chance that they'll spread but also harms the host. Consequently, microbes sometimes use very different methods to cause devastation. They can keep the carrier alive for a long time, which carries the potential to infect multiple victims over many months or years, as in the case of the HPV virus. Or they can kill and spread quickly, infecting dozens of new victims in the course of a day, as in the case of smallpox and cholera.
That a tiny microbe has the potential to alter the body and behavior of its host represents an enormous logistical feat. As scientists sequence the genomes of different species, they provide information on the relative size of the genetic blueprints that permit these organisms to function and give us a sense of how enormous the feat is. The numerical genome sizes of many cellular forms of life can range into the billions—humans, for example, have around three billion base pairs (i.e., bits of genetic information); corn has around two billion. Certain viruses like HIV and the Ebola virus, which use RNA rather than DNA for their genetic information, manage to live with an average of only ten thousand base pairs of genetic information, an incredible level of biological minimalism. How they manage to replicate with such a small amount of genetic information, let alone do something remarkably complicated, like altering the behavior of their hosts, is truly amazing.
Viruses manage to function with such few genes through a variety of tricks that allow them to maximize the impact of their diminutive genomes. Among the most elegant is a phenomenon called overlapping reading frames. As an analogy, take a poem of around thirteen thousand letters—say, T. S. Eliot's poem The Waste Land. It has roughly the same number of letters as the Ebola virus has base pairs. When you read The Waste Land, it has meaning, tempo, reference—all of the characteristics we normally expect from literature. In the same way, the genome of the Ebola virus has meaning, with base pair letters making up genes that get translated into the proteins that provide the virus with its capacity to function. If you take the first stanza of The Waste Land, around a thousand letters, and begin to read it starting with the second letter instead and move the first letters of the other words, it's a disaster. "April is the cruelest month" becomes "Prili sthec rueles tmonth." Nonsense.
Now imagine that embedded within the stanza was a second poem so that both readings, the one that starts with the first letter and the one that starts with the second letter, lead to fluent comprehensible verses. Now imagine that you took the same stanza and read it backward and that a third hidden stanza emerged from the same letters. This is precisely what viruses can do. A good challenge to poets (or perhaps computer scientists) would be to create such a stanza to see if they could be as creative as natural selection has been with viruses. Viruses with overlapping reading frames use the same string of base pairs to code up to three different proteins, an incredible genomic efficiency, which makes their small genomes pack a much larger punch.
Overlapping reading frames represent just one of a range of adaptations that viruses have to negotiate their worlds. Perhaps even more important for viruses is their capacity to generate genetic novelty. Viruses have a diverse toolbox for altering themselves. Among the most fundamental is simple mutation. No organisms have perfect fidelity. Any time a cell in our body or a bacterium divides to create daughter cells or a virus replicates in a host cell, errors creep in. This means that even in the absence of sexual mixing, offspring are never the same as their parents. Yet viruses have taken mutation to a completely new level.
Viruses have some of the highest mutation rates of any known organisms. Some groups of viruses, such as RNA viruses, have such high error rates that they approach a threshold where any higher level of mutation would make them effectively crash due to the loss of essential function from the resulting errors. While many of the mutations harm the new viruses, the high number of offspring that viruses produce increases the chances that some mutants survive and occasionally outperform their parents. This raises the chances that they will successfully evade the immune systems of their host, get the upper hand against a new drug, or gain the capacity to jump to a completely new host species.
Middle-school biology teaches us that life is made up of sexual or asexual organisms. Yet viruses and other microbes exchange genetic information in ways that should make us question our early textbooks. When two different varieties of virus infect the same host, from time to time they infect the same cell, setting the stage for such exchange. In these cases, viruses sometimes create mosaic daughter viruses, which include some genetic parts from one of the viruses and completely different elements from another. In the case of reassortment, entire gene segments are swapped between certain kinds of viruses. In recombination, genetic material from one virus is swapped into a second virus. Genetic mixing of both sorts provides viruses with a rapid and radical way to create novelty. As with mutation, the novel daughter viruses have new blueprints that occasionally help them survive and spread.
Our knowledge of microbes is still young. This vast unseen world is critical to our planet and our species, yet we understand very little about it. We've already discovered most of the plant and animal life on our planet, but we regularly discover brand-new microbes. Ongoing studies of the diversity of microbes in animals, plants, soils, and aquatic systems represent the tip of a very large iceberg. The millions of specimens that will result from these studies will catalyze our understanding of life. Among other things, the knowledge will help spark the development of new antibiotics. It will also help us forecast the next pandemic. The microbial world is the "new world," the last frontier of undiscovered life on our planet.
Copyright © 2011 by Nathan Wolfe
[1] Throughout this book, I'll generally refer to microbes rather than the more appropriate but clunky term microorganisms, which includes all microscopic organisms. Unless otherwise specified, the term microbe will be used as shorthand to refer to the full range of microscopic organisms whose groups include species that can infect and spread in humans, namely: viruses, bacteria (and their siblings, the archaea), parasites, and the enigmatic prions, all discussed in detail in chapter 1. While no doubt irritating to some of my microbiologist colleagues, who exclude parasites from the term microbe for sensible taxonomic reasons, and haven't quite decided what to do with prions, I hope they will excuse me in this attempt to increase the ease of reading for a general audience.
[2] There is some debate about whether viruses themselves should be considered living, while there is no debate about the other microbes: bacteria, archaea, or parasites, all of which are clearly living organisms. The debate, in my opinion, is a semantic and largely unimportant one. Viruses are completely dependent on other organisms for elements of their life cycle, but that is no different than the rest of known life forms, none of which, to my knowledge could live in a world devoid of other life. Either way, it is clear that viruses are part of the living systems of our planet, and for those intent on engaging in this debate, my reference to viruses as living can be interpreted in that way. I will use the same inclusive convention with prions, despite the existence of similar debates on them.
[3] In fact, the death rate for the 1918 H1N1 infection itself may be even lower than 2.5 percent, as many deaths were probably caused by secondary bacterial infections—deaths that could at least partially be prevented today due to antibiotic use. Deaths from H5N1, on the other hand, are largely due directly to viral disease.
[4]In the case of rabies, vaccine delivered in short order after infection can successfully prevent death, but without it death is largely inevitable.
[5] Like H5N1, the "swine flu" that began in 2009 suffers from terminology problems. Called H1N1/09 virus by the WHO and 2009 H1N1 influenza among other things by the CDC, here I will refer to it simply as H1N1, which is the commonly used shorthand among the scientists who study it. As with H5N1 and all influenza viruses, H1N1 has its ultimate origins in bird populations.
[6] There are some who consider Dmitri Ivanovski the "father of virology" because he did similar research with tobacco mosaic virus six years earlier. But perhaps because he wasn't the first to name the new entities (i.e., viruses) or did not as widely disseminate his findings as Beijerinck, he is not generally credited with their discovery.
[7] In addition to his pivotal work as the first virus hunter, creating the foundations of what would later become the field of virology, Beijerinck remains an unsung hero for those studying the relationships between plants and bacteria. Among other notable findings, he discovered nitrogen fixation, whereby bacteria living in the roots of legumes make nitrogen available to plants through a set of biochemical reactions critical for the fertility of agricultural soil systems.
[8] Among the most intriguing possibilities is that non-DNA/non-RNA forms of life, which originated completely independently of our own RNA/DNA-based life, might persist undetected on Earth. These life forms, referred to as shadow life, would almost certainly be microscopic. If discovered, they might best be described as aliens, and some believe that if we are to discover aliens within our lifetimes, looking on Earth will be our best shot.
Table of Contents
Introduction 1
Part I Gathering Clouds
1 The Viral Planet 19
2 The Hunting Ape 36
3 The Great Microbe Bottleneck 54
4 Churn, Churn, Churn 71
Part II The Tempest
5 The First Pandemic 93
6 One World 115
7 The Intimate Species 133
8 Viral Rush 153
Part III The Forecast
9 Virus Hunters 175
10 Microbe Forecasting 200
11 The Gentle Virus 218
12 The Last Plague 237
Sources 257
Acknowledgments 287
Index 293